Jennifer A. Doudna
Biographical
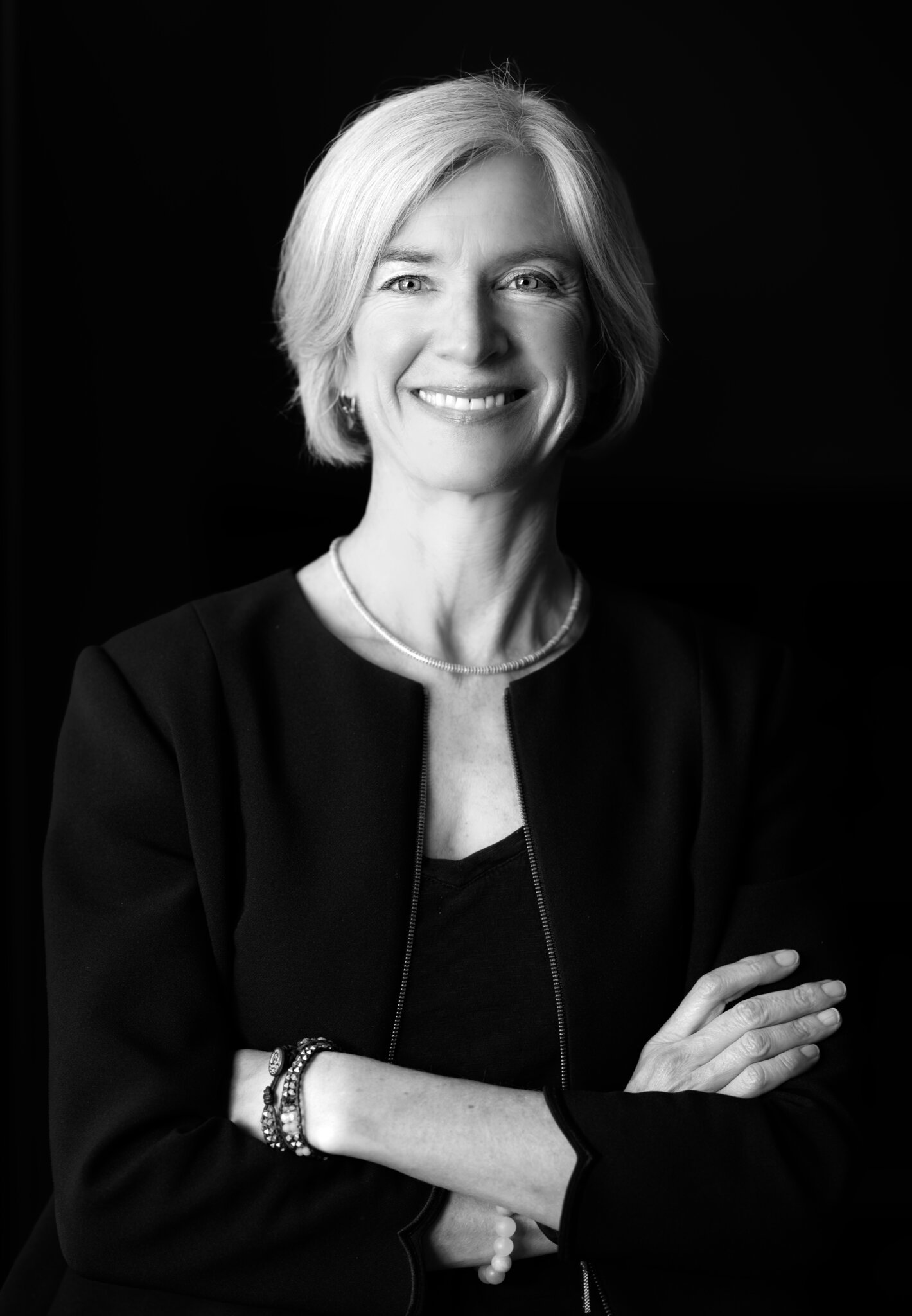
Iwas born on February 19, 1964 in Washington, D.C., the oldest of three sisters. My father Martin K. Doudna was a speechwriter for the Department of Defense at the time and my mother Dorothy taught in community college. My family, including my siblings Ellen and Sarah, moved to Ann Arbor where my father pursued a Ph.D. in literature at the University of Michigan. After earning his degree, he received a job offer from the University of Hawaii in Hilo and moved our family there in August 1971 when I was seven.
Growing up as a “haole” (Hawaiian slang for a non-native), I felt really alone and isolated at school. This “outsider” feeling drove me to take risks and prove doubters wrong, and later influenced my choices as a scientist. In my isolation, I sought solace in books that spurred me to learn more about the world around me and how I fit in. As I made friends and expanded my social life, I fortified my reading with nature walks, hikes, bicycle riding, and explorations of lava-flow caves. With its mix of volcanoes, forests and beaches, the “Big Island” of Hawaii provided a rich palette of biological diversity that inspired my first questions as to how so much diversity came to be.
In the sixth grade, I came across a book called The Double Helix by James Watson that my father had laid on my bed. It told how Watson, an American biologist, and Francis Crick, an English biochemist, led the discovery in 1953 of the “spiral staircase” structure of the DNA molecule, for which they received the 1962 Nobel Prize in Physiology or Medicine with Maurice Wilkins.
DNA is formed from chains of nucleotides, each of which contains one of four nitrogen bases – adenine, thymine, guanine and cytosine. All of the information for carrying out the essential processes of life is encoded in the sequences of these AGCT base letters. A string of base letter sequences that contains instructions for making a specific protein is called a gene. The Double Helix explained how DNA’s double-stranded helical structure – commonly depicted as a twisted ladder that enables the molecule to unwind − exposes its sequences of AGTC letters so that the genetic instructions they carry can be copied onto messenger RNA (mRNA). In the cells of eukaryotic organisms such as humans, mRNA carries these instructions from the nucleus out into the cytoplasm where transfer RNA (tRNA) uses the information to assemble amino acids into proteins.
In reading The Double Helix, I was captivated by how scientists, working collaboratively, were able to meticulously piece together and solve what had been one of biology’s most elusive puzzles.
Learning about the role played by Rosalind Franklin, the so-called “Dark Lady of DNA,” whose X-ray crystallography images exposed DNA’s helical shape, it struck me then for the first time that a woman could be a great scientist.
Despite my outstanding academic record, especially in math and science, my high school guidance counselor strongly discouraged me from pursuing a college major in chemistry, or even aspiring to be a scientist. Undeterred, I applied to and was admitted into Pomona College in California and enrolled in the fall of 1981 at the age of 17. I studied chemistry, despite briefly considering switching my major to French.
That summer I returned to Hilo and worked in the laboratory of Don Hemmes, a biology professor and longtime family friend. I was assigned to a small team investigating how a fungus, Phytophthora palmivora, infected papayas, which was a big problem for Hawaiian fruit growers. I learned to prepare samples for analysis in an electron microscope and follow the chemical changes that take place as the fungus advances through different stages of germination. The research revealed that calcium ions play a crucial role in the development of the fungus by signaling fungal cells to grow in response to nutrients. It was my first taste of the thrill of scientific discovery, an experience that I had read so much about, and that left me hungering for more.
In the summer of 1984, following sophomore and junior years that solidified my commitment to science, I was invited to work in the lab of my advisor, biochemistry professor Sharon Panasenko. I was tasked with growing soil-based bacteria in such a way where we could study the chemical signaling that enables the bacteria to self-organize into colonies when starved for nutrients. My method of growing the bacteria in large baking pans instead of conventional Petri dishes was acknowledged in a paper published by Sharon in the Journal of Bacteriology, the first time my name appeared in a scientific journal.
I graduated from Pomona College in the spring of 1985 as the top student in chemistry. I remain grateful to Pomona for a liberal arts education that exposed me to so many ideas that I otherwise might have never had come into contact with and that were key to my later success.
Upon the urging of my father, I applied to graduate school at Harvard Medical School, where I enrolled in the fall of 1985. In 1986, I elected to do my dissertation work in the lab of Jack Szostak (2009 Nobel Prize laureate in physiology or medicine with Elizabeth Blackburn and Carol Greider) who discovered how telomere caps prevent chromosomes from breaking down.
Jack was in the process of switching his research focus from DNA to RNA and the role it played in the origin of life on Earth. He suspected RNA preceded DNA and encouraged me to be his first graduate student to explore this idea.
In retrospect my decision to concentrate on RNA makes perfect sense, but at the time turning away from DNA was a bold and risky move. The public spotlight then was shining on the Human Genome Project, an international effort to map and sequence all the base letters in the human genome (an organism’s full complement of DNA).
Billed as “the Holy Grail of Biology,” the Human Genome Project promised to revolutionize medical diagnostics and treatments with the wealth of genetic information it would provide. Mapping and sequencing DNA garnered headlines and funding, but we saw the immense value to be gained from a better understanding of the multipurpose RNA molecule in all its many forms.
Jack and I agreed that the potential for RNA to have played a starring role in the origin of life hinged upon whether RNA molecules are able to replicate themselves. To answer this question, we reengineered a self-splicing RNA intron (a segment of a DNA or RNA molecule that does not code for proteins) into an RNA enzyme (a protein catalyst) that could splice together a copy of itself. This demonstrated that RNA could function as a polymerase, an enzyme that promotes the formation of molecules such as DNA or RNA. We published the results of the study in 1989 in the journal Nature in a paper titled “RNA-catalyzed synthesis of complementary-strand RNA,” marking the start of my journey in RNA research.
After receiving my Ph.D. in 1989, I continued my research on self-replicating RNA molecules in Jack’s laboratory as a postdoctoral fellow, but my curiosity grew around what sort of molecular structure would enable RNA to replicate itself. It was one of biology’s toughest challenges – determining the molecular structures of RNA enzymes and other functional RNA molecules.
In the summer of 1991, I relocated to the University of Colorado in Boulder to work in the laboratory of Tom Cech, who two years earlier had won the Nobel Prize in Chemistry with his collaborator Sidney Altman. In the 1980s they discovered that RNA not only serves as a genetic messenger but can also function like an enzyme. They dubbed these catalytic RNAs “ribozymes” by combining “ribonucleic acid” with “enzyme.” Their ribozyme was the same self-replicating RNA enzyme that Jack and I would later reengineer.
As a research fellow in Tom’s laboratory, I used X-ray crystallography to image the structure of RNA enzymes, similar in principle to how Rosalind Franklin imaged the structure of DNA. For this work, I teamed up with a graduate student named Jamie Cate who had been using X-ray crystallography to study protein structures. Although we were able to successfully crystalize RNA enzymes for imaging, exposure to X-rays quickly destroyed the crystalline structure.
It was a fortuitous meeting with Yale University biochemists Thomas Steitz (2009 Nobel Prize laureate in chemistry with Venkatraman Ramakrishnan and Ada Yonath) and Joan Steitz, a husband-and-wife team on sabbatical in Boulder for a year, where I learned of their technique for the cryogenic cooling of crystals prior to X-ray imaging. Flash-freezing crystals in liquid nitrogen preserved their crystalline integrity even when irradiated with X-rays. Steitz would share the 2009 Nobel Prize in Chemistry for his work in determining the structure of ribosomes, the macromolecules in a cell’s cytoplasm that use RNA’s genetic messages to synthesize proteins.
Eager to use cryo-cooling technology, I accepted an appointment at Yale in 1994 as an assistant professor of molecular biophysics and biochemistry, with Jamie Cate accompanying me as a graduate student in my new lab. With a technique devised by Jamie, we used X-ray crystallography to produce electron-density maps that enabled them to determine the location of every atom in a self-splicing RNA enzyme. With this information, we constructed structural models of the molecule, similar to what Watson and Crick did with DNA.
Just as the double-helix structure revealed how DNA is able to store and transmit the genetic code, the structural models that my team and I built showed how RNA is able to function as an enzyme capable of slicing, splicing and replicating itself, just as the double-helix structure revealed how DNA is able to store and transmit the genetic code. Tom and I were the principal investigators and Jamie Cate was the lead author on a 1996 paper published in Science titled “Crystal Structure of a Group I Ribozyme Domain: Principles of RNA Packing.” The paper is considered a scientific landmark for providing the first detailed look at a large-structured ribozyme. We hoped then that our discovery would provide clues as to how scientists might be able to modify the ribozyme to repair defective genes in the future.
In 1997 I accepted an appointment as a Howard Hughes Medical Institute (HHMI) investigator. In 2000 I was named the Henry Ford II Professor of Molecular Biophysics and Biochemistry and elected into the National Academy of Sciences. That summer I married my research partner Jamie Cate and we had a son whom we named Andrew two years later. It was also in 2002, after Andrew’s birth, that Jamie and I accepted appointments as professors in the College of Chemistry at the University of California, Berkeley (UC Berkeley). We also became faculty scientists at the Lawrence Berkeley National Laboratory (Berkeley Lab), which gave us access to the Advanced Light Source, one of the world’s premier sources of X-rays for crystallography research.
In the fall of 2002, soon after I started my work at UC Berkeley, there was a deadly outbreak in China of Severe Acute Respiratory Syndrome (SARS) caused by an RNA-based virus. The outbreak motivated me to pivot to RNA interference, a phenomenon that plays a fundamental role in a number of important functions, including how the human immune system fights off viral infections. RNA interference silences unwanted genetic messages, thereby blocking the production of the proteins they code for.
Using the powerful beams and sophisticated instrumentation at the Advanced Light Source, a synchrotron accelerator optimized for X-ray and ultraviolet light research, my lab and I produced crystallography images from which we determined that an enzyme known as Dicer functions as a molecular ruler to snip double-stranded RNA and initiate the process of RNA interference. We found that the molecular structure of Dicer features a “clamp” at one end to grab hold of a double-stranded RNA molecule, and a “cleaver” a set distance away at the other end to snip it.
Our findings set the stage for understanding how Dicer enzymes are involved in other phases of the RNA interference pathway serving as a guide to redesigning RNA molecules that direct specific gene-silencing pathways. Our work later led to a call from UC Berkeley microbiologist Jillian Banfield who first introduced me to the term “CRISPR.”
Jill was studying the genomics of microbes that live in extreme environments as a means of finding better ways to clean up polluted sites, and repeatedly encountered a unit or length of DNA base letter sequences known as CRISPR, or Clustered Regularly Interspaced Short Palindromic Repeats, which plays a role in the defense mechanisms employed by microbes such as bacteria and archaea for protection from viruses and invading strands of nucleic acid known as plasmids. Usually located on a microbe’s chromosome, a CRISPR unit is made up of base sequences called “repeats” and “spacers” that separate the repeated sequences. Interested in understanding how a combination of CRISPR and a complex of adjacent enzymes dubbed “Cas” for CRISPR-associated proteins allowed microbes to utilize small customized RNA molecules (crRNA) to protect themselves through a gene-silencing process, Jill found my lab’s website from a Google search and suggested a collaboration to learn how the CRISPR-Cas immune system might work through RNA interference.
A conversation over tea at a local Berkeley café followed, where we discussed this new, mysterious biological function of CRISPR and the possibility that it was the bacterial equivalent to RNA interference. While hypotheses about CRISPR had been floated, no one had yet conducted the experiments to prove or disprove those theories.
In 2008, Jill and I, along with Mark Young at Montana State University, organized the first international conference on CRISPR research, which was held in Berkeley. That same year, research out of Northwestern University had revealed that the CRISPR-Cas system did not work through RNA interference, but instead targeted the DNA of an invader. The implication of this discovery was highly significant. If CRISPR-Cas was identifying, targeting, and cutting invasive DNA, then it had potential as a DNA editing tool. However, crucial experimental information was missing, especially as to how the CRISPR-Cas system would be able to recognize and cut out unwanted DNA.
My Doudna Lab team began filling in this missing information by first developing a purification technique that provided them with highly concentrated samples of the Cas proteins they wanted to study. With this technique, we first studied Cas1, which we discovered is able to cut up DNA in a way that helps with the insertion of new snippets of foreign DNA into a CRISPR unit during the immune system’s memory-forming stage. It brought us a step closer to understanding how CRISPR steals bits of DNA from attacking phages and works that genetic information into its own, laying the groundwork for the targeting and destruction phases of the immune response.
In 2010 we used X-ray crystallography beamlines at the Advanced Light Source to produce the first atomic-scale crystal structure model of Cas6f, discovering that like Cas1, it functions as a chemical cleaver. However, we found that the job of Cas6f is to specifically and methodically slice long CRISPR RNA molecules into shorter chunks that can be used to target foreign DNA.
Our model showed that when a microbe recognizes it has been invaded by foreign DNA, it incorporates a small piece of that foreign DNA into one of its CRISPR units, which is then transcribed as a long RNA segment called the pre-crRNA. Cas6f cleaves this pre-crRNA within each repeat element to create short crRNAs containing sequences that match portions of the foreign DNA forCas proteins to use to bind the foreign DNA and silence it. These research results were reported in the journal Science in a paper titled “Sequence- and structure-specific RNA processing by a CRISPR endonuclease.”
In 2011 I met Emmanuelle Charpentier at the annual American Society for Microbiology meeting in Puerto Rico. A French biochemist, microbiologist, and geneticist, and one of the world’s top CRISPR-Cas researchers, Emmanuelle was at the time studying Cas9 in a CRISPR system known as type II at the Laboratory for Molecular Infection Medicine at Umeå University in Sweden. Her work had shown that in the human pathogen Streptococcus pyogenes, crRNAs could only be produced in the presence of a second CRISPR RNA molecule, or tracrRNA for trans-activating crRNA, and that CRISPR systems only need Cas9 to acquire immunity to viruses targeted by crRNAs.
Emmanuelle and I embarked on a collaboration to investigate how Cas9 and crRNAs function in the CRISPR microbial immune system and whether crRNA and tracrRNA could be linked into a single chimeric CRISPR RNA molecule to make the system easier to manipulate.
With Martin Jinek and Michael Hauer from my lab and Krzysztof Chylinski and Ines Fonfara from Emmanuelle’s, we unraveled the components of the CRISPR-Cas9 assembly. In our discovery that Cas9 requires binding to a molecular complex of tracrRNA and crRNA which then identifies and guides the Cas9 to the invasive DNA for cleaving, we realized that the tracrRNA and crRNA complex is programmable.
The next step was to engineer a single-guide RNA (sgRNA) molecule that would have the guide information on one end and the binding handle on the other. Such a system would provide a straightforward way to cleave any desired stretch of DNA sequences in a genome. New genetic information could then be introduced into the genome using well established cellular DNA recombination technology.
The results of this momentous study were published in Science on August 17, 2012 (it first appeared online June 28, 2012). The paper was titled “A Programmable Dual-RNA–Guided DNA Endonuclease in Adaptive Bacterial Immunity.” Emmanuelle and I were the principal investigators; the co-authors were Martin Jinek, Krzysztof Chylinski, Ines Fonfara, and Michael Hauer.
Our paper showed that an immune system evolved over eons by bacteria and other microorganisms to defend themselves against viral and other invasive DNA could be adapted into a relatively easy-to-use “CRISPR genome editing” technology for rewriting the genetic code within the cells of any organism, including humans, with unparalleled efficiency and precision.
It was an incredible, precious time of pure joy to discover that bacteria had found a way to program a warrior protein to seek and destroy viral DNA, and fortunate, even miraculous, that we could repurpose this fundamental property for an entirely different use. The joy of discovery was a feeling just like I’d felt in Don Hemmes’s lab all those years before in Hawaii.
In 2014, I founded the Innovative Genomics Institute (IGI) with Jonathan Weissman to realize the potential of CRISPR genome editing in human health, climate, and agriculture. The IGI, of which I am president, is made up of researchers at UC Berkeley and the University of California, San Francisco, who are developing foundational CRISPR technologies to advance genome engineering innovations for the benefit of humanity that are accessible and affordable to all. We continue to build upon what started as our curiosity-driven, fundamental discovery project into strategies to help improve the human condition.
One of the early initiatives of the IGI centered around expanding access to treatments for sickle cell disease. By this time, I had additionally launched my first company, Caribou Biosciences, and would come to co-found companies Editas Medicine, Intellia Therapeutics, Mammoth Biosciences, and Scribe Therapeutics to bring CRISPR from the lab into the clinic in the form of CRISPR-based therapeutics and diagnostics.
I accepted the positions of Li Ka Shing Chancellor’s Chair at UC Berkeley, senior investigator at the Gladstone Institutes, and adjunct professor of cellular and molecular pharmacology at UC San Francisco, persisting with my research on the exploration of delivery techniques for CRISPR-based therapies, the development of next-generation CRISPR diagnostics, and continued investigations into the structure and mechanism of CRISPR-Cas systems. Our ability to harness the immense “dual use” potential of CRISPR led to my decision to actively engage in and initiate the public discourse on the ethical use and responsible regulation of CRISPR, particularly in the case of human germline editing.
In 2015 I first called for a moratorium on the use of CRISPR in the human germline and arranged a meeting with 20 other researchers with the goal of discussing the ethics of germline gene editing. Modeled after the 1975 Asilomar conference that put forward guidelines for research on recombinant DNA and co-organized with two of its key organizers Paul Berg and David Baltimore, the discussions we conferred at the Napa Valley meeting were summarized in a report later published in Science. In it, we outlined the implications of human germline editing and ultimately called for a prudent path forward, including clear international guidelines, over instituting a moratorium.
I was also one of the organizers of the International Summit on Human Gene Editing in December 2015, the first international symposium at the National Academy of Sciences on the societal and ethical use of CRISPR technology. At the event, we further discussed the ethical considerations of safely applying CRISPR technology and came to a consensus similar to that which resulted from the Napa conference – that certain conditions should be met before human germline editing was permitted. As CRISPR began to enter the mainstream conversation, I detailed the ethical quandaries we faced and our rationale for calling on ongoing input from scientists and bioethicists as well as broader public discussion in my 2017 book A Crack in Creation: Gene Editing and the Unthinkable Power to Control Evolution co-authored with Samuel Sternberg. I had hoped that the steps Sam and I described would deter any premature attempts to perform inheritable gene editing but just one year later the type of reckless experiment that I had feared might happen did.
At the 2018 Second International Summit of Human Genome Editing in Hong Kong, news of the world’s first “CRISPR babies” born in China – a medically unnecessary, illegal human experimentation – propelled us into a new era. Along with my colleagues from the National Academies of Sciences and Medicine and the Royal Society, many of whom were participants at the 2015 Napa Valley conference, I maintained that the risks of germline editing remained too great to permit gene editing in embryos, egg cells, or sperm cells. Back in the U.S., I met with a number of senators to discuss the safest path forward for CRISPR technology – and its future impact on our health – and continued to guide conversations on the necessary regulations including the international commissions that have since been convened by the National Academies and by the World Health Organization.
In early 2020, I expanded my work with CRISPR as called for by the global COVID-19 pandemic. My colleagues at the IGI and I launched an automated coronavirus clinical testing laboratory in March 2020 built out of our research facilities over the course of just three weeks. Our group has since provided critical testing services to thousands across local and state communities. We also introduced a new initiative to develop a rapid CRISPR-based, point-of-need diagnostic test in addition to approximately two dozen additional research projects. We realized the need to further enable acts of scientific collaboration and innovation that would lead us out of the pandemic, releasing a roadmap detailing the transformation of our nonclinical labs into the testing facility and making all COVID-19 project-related intellectual property open source.
Our group continued to pursue genome engineering research that could elevate the standard of care for patients around the world and of the highest, currently unmet need. Our efforts to date, from Emmanuelle and my co-discovery of CRISPR to the genome editing revolution happening now, were chronicled by bestselling author and historian Walter Isaacson in his book The Code Breaker: Jennifer Doudna, Gene Editing, and the Future of the Human Race in March 2021. Shortly after, the IGI, leading a consortium of scientists and physicians across UC Berkeley, UCSF, and the University of California, Los Angeles, began the first FDA-approved clinical trial of a CRISPR-based therapy for directly correcting the genetic mutation that causes sickle cell disease. We are developing the sickle cell therapy, and future CRISPR gene therapies, to eventually treat disease from within the human body (in vivo) and extend to blood cancers, immunological conditions, and additional rare diseases that presently cannot be addressed. By improving our ability to rewrite the code of life and supporting the type of fundamental scientific research that made our discovery of CRISPR possible, I believe they soon can be.
© The Nobel Foundation 2024
Nobel Prizes and laureates
See them all presented here.